A physics phenomenon under the Antarctic ice reveals a rarely seen force
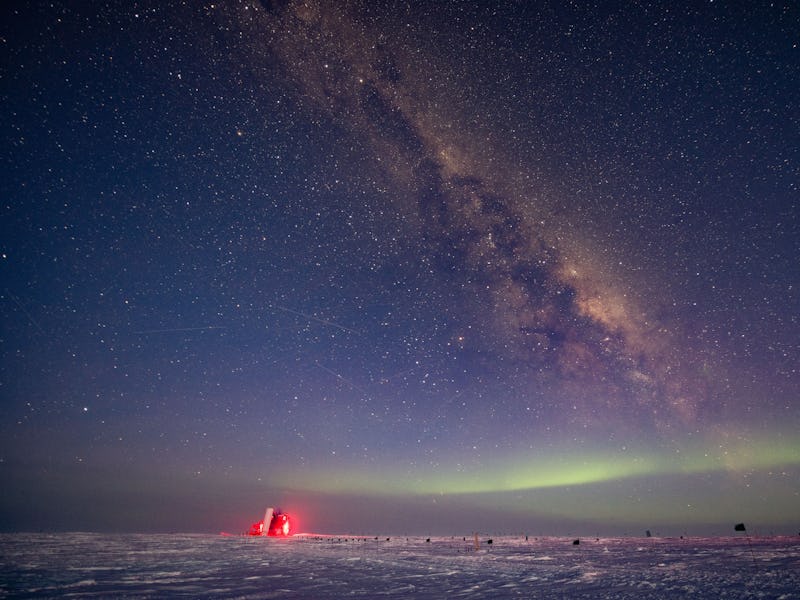
In 2016, Earth’s South Pole was struck by a ghostly particle from some unknown corner of the universe.
This antineutrino — the neutrino’s antimatter twin — collided with an electron at nearly the speed of light, and the ensuing particle shower lit up a network of detectors buried beneath the ice at an observatory in Antarctica appropriately named IceCube.
Within that particle shower, astronomers found signs of something never seen before in any observatory.
The collision spun off a W-minus boson, an elementary particle that carries the so-called “weak force,” one of four fundamental forces in physics.
This phenomenon is called a Glashow resonance. And while scientists had first predicted its existence more than 60 years ago, this is the first time they’d actually seen it.
“This was really something very new,” University of Wisconsin physicist Francis Halzen, IceCube’s principal investigator, tells Inverse. “We have seen neutrinos of the same energy before, but we have never seen one that produces a W-minus boson. It’s something very different from what we normally look at, and so we studied this event to death.”
The new study, authored by some 300 physicists from the IceCube collaboration, was published Wednesday in the journal Nature.
What’s new — Neutrinos are a fundamental subatomic particle with no electrical charge and minuscule mass. Fundamental particles are the building blocks of matter, and they can’t be broken down into other particles. Those ghostly qualities mean that neutrinos rarely interact with matter, making them extremely difficult to detect.
That’s why astronomers built the IceCube Neutrino Observatory in Antarctica. There, IceCube makes use of thousands of sensors that extend nearly a mile below the ice. Typically, neutrinos pass through Earth at the speed of light, unbothered by our mass. Astronomers say as many as 100 trillion neutrinos pass through our bodies every second, most going right through unnoticed.
But on rare occasions, a neutrino will collide with another particle inside an atom. These events release a cascade of other subatomic particles along with a light pulse that IceCube’s detectors can see since they’re buried beneath the ice.
That’s what happened in 2016. But it didn’t take astronomers long to realize something was different about this detection. And as they worked through the details, they realized that they’d seen this Glashow resonance, and hundreds of IceCube scientists started to get excited.
To measure neutrinos, the IceCube detector has to dig deep into the ice, which also helps rule out interference from above ground.
Here’s the background — The idea that a high-energy neutrino striking an electron could create a boson, or force-carrying particle, was first put forth back in 1960 by Nobel Prize-winning physicist Shelly Glashow. He was among the handful of scientists working on what would eventually become the Standard Model of Physics, the theory that classifies all known elementary particles and describes three of the four fundamental forces, which are the strong, weak, and electromagnetic forces.
Today, the Standard Model represents physicists’ best understanding of our universe at the smallest scales. However, it’s incomplete. The Standard Model can’t explain things like dark matter and dark energy, which make up most of our universe. It also doesn’t include gravity, the fourth fundamental force. So, any concrete evidence that the Standard Model is right — or wrong — can help physicists figure out if they’re on the right track.
The IceCube team knew that if they could verify this long-predicted Glashow resonance, it would also provide a crucial confirmation that the Standard Model still holds even at the highest energies.
Those high stakes and the level of complexity meant it would ultimately take the physicists nearly five years to publish their discovery, as their conclusions needed to be airtight.
The IceCube collaboration hunts for some of the most elusive particles in the universe by looking for miniscule reactions in Antarctic ice.
How they did it — As Halzen explains, particle colliders like the Large Hadron Collider in Switzerland have multiple detectors, so if one instrument sees something, the others can verify the finding.
But IceCube doesn’t have that luxury. As a neutrino passes through the pristine Antarctic ice, there’s just an infinitesimal chance that it will collide with another particle. But by placing sensors across a cubic kilometer of ice, IceCube drastically increases its chances of actually catching these events in action. However, its unique position means it’s also the only instrument on Earth that could’ve detected this event. No one else can check their work.
“We are extremely conservative because if we start publishing things and have to take them back, nobody will listen,” Halzen says.
However, Halzen didn’t hesitate to send the image to Glashow soon after the initial discovery.
“We’ve seen [the W boson], but we are 30 years too late,” Halzen joked with the now 88-year old physicist. Researchers at CERN had already confirmed the W boson’s existence back in the 1980s, but the particle collider couldn’t reach energies high enough to produce the Glashow resonance.
“It's too bad we missed it because the person who first detected it won the Nobel Prize,” Halzen says.
IceCube could change our ideas about the cosmos
Why it matters — The nature of the interaction also let IceCube’s scientists discover this neutrino’s “flavor,” something that’s also only previously been possible in particle colliders.
Neutrinos come in different varieties, which physicists call flavors. Rather than vanilla or chocolate, there are electron neutrinos, like the one involved here, as well as muon neutrinos and tau neutrinos. IceCube team member Doug Cowen, a physicist at Penn State, tells Inverse knowing this particle’s flavor may prove to be the most important part of the discovery.
Physicists predict that the cosmos should create an equal number of all of these flavors. And that’s what experiments have shown in the controlled environments of colliders here on Earth. So, IceCube should detect an equal number of electrons, muons, and tau neutrinos.
If it turns out this ratio isn’t perfect, it would reveal some strange things about the universe. Maybe neutrinos aren’t made the way physicists predict. Or perhaps something is stealing off certain neutrinos as they travel the cosmos.
There are some researchers who think certain neutrino flavors could be deterred by things like unseen dimensions and something called “spacetime foam,” where microscopic oscillations could be jiggling the very fabric of the universe.
“Unfortunately, it's a little hard with the stats we have now to disentangle whether something is going on with the source, or if something is happening on the way to Earth,” Cowen says.
What’s next — IceCube is currently lobbying to get a roughly $300 million upgrade and expansion in the years ahead, called IceCube-Gen2.
The current observatory is using technology that’s now 15 years old, and updates to its sensors and the strings that connect them will let the detector better see where neutrinos are coming from in Earth’s night sky. In theory, this bigger, better IceCube would detect Glashow events every year, instead of one every decade. And as researchers expand the catalog of these particles and their flavors, they’ll know whether this perfectly even ratio holds up in the real world.
This second-generation upgrade would also help astronomers track down the extreme objects that create such high-energy particles in the first place.
“[This discovery] is important not just because it confirms the Standard Model,” Lu Lu, a University of Wisconsin physicist and one of the study’s main authors, tells Inverse. “It is also extremely interesting for understanding the properties of the source environments.”
For all the observations IceCube has made, scientists still can’t pinpoint where these astrophysical neutrinos are coming from. They could be streaming from supermassive black holes, colliding stars, and more.
But astronomers won't know for sure until they can pinpoint the direction the particles are coming from and partner with traditional optical telescopes to collect images. The massive Vera C. Rubin Observatory will soon open in Chile, and it will image the entire visible sky every few nights. If IceCube gets an upgrade and starts detecting more Glashow events with better accuracy, astronomers will be able to marry those observations with images from the Rubin telescope and finally crack the mystery.
Abstract: The Glashow resonance describes the resonant formation of a W− boson during the interaction of a high-energy electron antineutrino with an electron1, peaking at an antineutrino energy of 6.3 petaelectronvolts (PeV) in the rest frame of the electron. Whereas this energy scale is out of reach for currently operating and future planned particle accelerators, natural astrophysical phenomena are expected to produce antineutrinos with energies beyond the PeV scale. Here we report the detection by the IceCube neutrino observatory of a cascade of high-energy particles (a particle shower) consistent with being created at the Glashow resonance. A shower with an energy of 6.05 ± 0.72 PeV (determined from Cherenkov radiation in the Antarctic Ice Sheet) was measured. Features consistent with the production of secondary muons in the particle shower indicate the hadronic decay of a resonant W− boson, confirm that the source is astrophysical and provide improved directional localization. The evidence of the Glashow resonance suggests the presence of electron antineutrinos in the astrophysical flux, while also providing further validation of the standard model of particle physics. Its unique signature indicates a method of distinguishing neutrinos from antineutrinos, thus providing a way to identify astronomical accelerators that produce neutrinos via hadronuclear or photohadronic interactions, with or without strong magnetic fields. As such, knowledge of both the flavour (that is, electron, muon or tau neutrinos) and charge (neutrino or antineutrino) will facilitate the advancement of neutrino astronomy.
This article was originally published on